1.IntroductionFollowing their discovery in the 1950s, corticosteroids have been widely used for inflammatory skin disease.1 As anti-inflammatory agents that generally reduce inflammation and immune response, their therapeutic effectiveness and efficacy in treating various skin diseases, such as atopic dermatitis, asthma, and rheumatoid arthritis, have been clinically verified,2–4 and currently, these agents are prescribed for the treatment of skin diseases. The corticosteroid acts in the body through the following mechanism: vasoconstriction, anti-inflammatory, immunosuppressive, and anti-proliferative responses.5 For example, the corticosteroid reduces erythema by constricting the capillaries in the shallow dermis through the inhibition of the vasodilators’ secretion (e.g., prostaglandin), thereby exhibiting anti-inflammatory action.6,7 This vasoconstrictor ability—the ability to whiten the skin—determines its effectiveness. Therefore, unlike drugs in other therapeutic classes, such as antifungal and antibacterial agents, topical corticosteroid products are graded and classified according to their effectiveness. However, skin whitening, i.e., the blanching effect, is an indirect indicator of the extent to which corticosteroids cause vasoconstriction, and deviations may arise depending on the difference in skin thickness between individuals. Notably, the effectiveness of corticosteroids can be assessed by quantifying the mechanism through which they constrict capillaries and eventually whiten the skin; thus, several techniques have been attempted to characterize the blanching effect more accurately. Initially, reflectance spectrometry, laser-Doppler velocimetry, and thermography have been employed and refined for the assessment of the effectiveness; however, these techniques were insensitive, difficult to implement, and less precise for quantitative appraisal.8,9 Recently, colorimetry of the skin-blanching response via a portable chromameter has emerged as a frequently used strategy in medical fields for tracking the progress of the blanching effect.10,11 However, this colorimetric-based vasoconstrictor assay is entirely based on visual evaluation, and it is still difficult to standardize due to inaccurate quantification.12,13 Additionally, deviations may arise due to differences in skin color for each patient. Above all, a majority of the existing methods have indirectly quantified the blanching effect caused by vasoconstriction rather than characterizing the vasoconstriction itself. Thus, the direct quantitative monitoring of vascular changes has not yet been attempted. Photoacoustic (PA) imaging—a specialized tool for visualizing blood vessels—is a promising candidate for assessing vasoconstriction. PA imaging is a hybrid imaging technique that detects ultrasound (US) waves generated through instantaneous heating and thermal expansion by irradiating optical absorbers with a pulsed laser. In particular, the hemoglobin contained in blood is an endogenous chromophore that is more sensitive than other biological tissues in visible wavelengths; thus, PA imaging can noninvasively visualize the blood vessels without the need for any contrast agent.14–16 In addition, owing to the advantageousness of the three-dimensional (3D) data acquired through PA imaging, this technique is widely used for quantitative volumetric analysis in diverse clinical fields.17–22 Among the currently available systems for PA imaging, PA microscopy (PAM) yields high-resolution images with a resolution of a few micrometers by tightly focusing light and/or acoustic waves on a single spot.23–36 Furthermore, the PAMs have achieved high-speed capability due to the introduction of micro-electro-mechanical system (MEMS)-based mirror scanning,37 and since then, various high-speed water-immersible scanners such as galvanometers and polygon-mirror scanners have been developed to increase the imaging speed of PAMs.38,39 Notably, this imaging technique has been used to visualize and monitor microvessels in various studies focusing on wound healing, stimulus response, drug delivery, and regenerative medicines.40–44 In this study, we propose a new approach to assess the vascular effects of corticosteroids using PA imaging. We used an optical-resolution PAM (OR-PAM) system in which optical focusing is much tighter than acoustic focusing because this system has better spatial resolution than acoustic-resolution PAM (AR-PAM) within the optical diffusion limit, allowing us to observe capillary changes with high resolution.45 The PA blood vessel images were obtained from a total of 24 mice, six from each of the following groups: (1) corticosteroid subcutaneous injection, (2) corticosteroid topical application, (3) nonsteroidal topical application, and (4) no injection or topical application. We longitudinally monitored vasoconstriction induced by the corticosteroids, quantified the extent of vasoconstriction depending on the skin layers, and compared the changes in blood vessel density between the four groups. Considering the remarkable results obtained via observing and quantifying the corticosteroids-induced vasoconstriction in the skin, we believe that high-resolution PAM has great potential for evaluating the effectiveness of corticosteroids. 2.Methods2.1.Optical-resolution Photoacoustic MicroscopyFigure 1(a) shows the OR-PAM system. The system is equipped with a 532-nm nanosecond pulsed laser (AWAVE532-1W-10K, Advanced Optowave, New York) for optical excitation. The laser spot size of our OR-PAM system is , and the laser repetition rate for imaging is 10 kHz. The laser beam is delivered to the PAM system using a single-mode fiber (P1-460B-FC-1, Thorlabs, New Jersey). The single-mode beam from the fiber is collimated using a reflective collimator (RC08FC-P01, Thorlabs, New Jersey) and is focused under the skin surface via an objective lens (AC127-050-A, Thorlabs, New Jersey) and a water-immersible MEMSscanning module (OptichoM-MS, Opticho, Republic of Korea). In the scanning module, the laser beam is reflected by an opto-ultrasound beam combiner (OUC) and a MEMS scanner to irradiate the imaging target, thereby generating PA waves [Fig. 1(b)]. The PA waves generated at the target pass through the OUC and are subsequently acquired using a US transducer (V214-BC-RM, Olympus NDT, Massachusetts), which is attached to the beam combiner to convert the PA waves into PA electrical signals. The acquired PA signals are amplified using a 50-dB amplifier (PE15A1013, Pasternack Enterprises, California). The amplified signals are digitized and thereafter transferred to a personal computer using a digitizer (ATS9350, Alazar Technologies, Quebec, Canada) at a sampling rate of . Additionally, the aforementioned components are installed on stacked motorized linear stages (L-509, Physik Instruments, Germany) to expand the field of view. A -axis manual stage for the target is utilized to adjust the distance between the system and target for focus optimization. All 3D data were post-processed and analyzed through MATLAB R2021a (MathWorks, Massachusetts) and 3D Photoacoustic Visualization Studio (3D PHOVIS).46 The lateral and axial resolutions of the OR-PAM system are 5 and , respectively.47 The maximum penetration depth of the OR-PAM system is from the epidermis, and the temporal resolutions for B-scan and volumetric imaging are 80 ms and 48 s. Fig. 1(a) Graphical representation of the optical-resolution PA microscopy (OR-PAM) system based on a micro-electro-mechanical system scanner. (b) Optical and US beam paths in the magnified view of the red dashed box in (a). (c) A mouse mounted on the -axis stage. The yellow box indicates the region of interest (ROI). OL, objective lens; OUC, opto-ultrasound beam combiner; WT, water tank; UT, ultrasound transducer; and MEMS, micro-electro-mechanical system. ![]() 2.2.Animal Preparation and Group CategorizationAll experimental procedures for in vivo experiments were approved by the Institutional Animal Care and Use Committee of the Pohang University of Science and Technology. To observe the vasoconstrictive effect of corticosteroids, 24 six-week-old nude mice were categorized into four groups as follows:
2.3.Experimental Procedure for in Vivo PA MonitoringA mouse was initially anesthetized with a gas mixture of 2.5% isoflurane at a flow rate of 1 l/min using an isoflurane vaporizer (Matrx VIP 3000, Midmark). During the experiment, a mouse in the supine position was anesthetized with 1.5% isoflurane, and its left leg was placed onto a customized leg holder affixed onto the -axis stage [Fig. 1(c)]. Subsequently, the following procedures were performed for each group:
The amount of hydrocortisone used for groups (1) and (2) was calculated in proportion to the body weight of the six-week-old nude mice compared to the amount of hydrocortisone used in humans in clinical practice.48–51 Additionally, for hydrocortisone subcutaneous injection, the amount of solution was experimentally optimized in both aspects. First, PBS should sufficiently dissolve the hydrocortisone, and second, it should not exceed an excessive amount that causes the mouse’s thigh to swell and interfere with image acquisition. After the procedures for each group, the mouse’s thigh was covered with US gel and subsequently contacted with a thin plastic membrane under a water tank to smoothly propagate the PA waves. The scanning module was submerged in water to ensure that the preset confocal point was focused on the vascular networks in the thigh. Thereafter, PA 3D data were continuously acquired at a time interval of 10 min for 60 min to monitor the changes in vascular density. The laser energy measured on the skin surface was , which was less than the maximum permissible skin exposure () specified by the American National Standards Institute. In addition, the biosafety of our experimental procedure involving laser irradiation after topical steroid treatment has been validated by clinical studies of patients applying topical steroid treatment using clinical PA imaging systems.52,53 3.Results and Discussion3.1.Layer-by-layer Vascular Representation from Volumetric PA DataVolumetric PA data ( along the , , and axes at 5-, 10-, and intervals, respectively) were acquired at the site of the mouse thigh. Figures 2(a) and 2(b) illustrate the 3D-rendered PA amplitude and depth-encoded images, which render a clear representation of the blood vessels and their networks owing to the higher optical absorption in hemoglobin than in other biological tissues. The PA data were segmented to characterize the skin layers where the administered corticosteroids dominantly acted upon the blood vessels. Generally, the skin layers comprise the epidermis, papillary dermis, reticular dermis (RD), and hypodermis (HD) along the skin depth, and the blood vessels are distributed differently depending on the skin layer [Fig. 2(c)]. Although the epidermis does not contain blood vessels, the capillary blood vessels are densely distributed in the papillary dermis, and relatively large blood vessels with arteriole–venule pairs are present in the HD.54–58 Moreover, the RD includes small blood vessels vertically connecting the blood vessels in the papillary dermis (PD) and HD.59 Observably, these features are represented in both the PA maximum amplitude projection (MAP) image along the direction [e.g., “A” view shown in Fig. 2(c)] and its depth-dependent histogram of PA amplitude [Figs. 2(d) and 2(e)]. Meanwhile, Fig. 2(d) is a projection of all 2D cross-sectional images in volumetric data, but it contains artifacts due to the mouse’s heartbeat and respiration resulting in the unsmooth skin surface. Although it takes 48 s to acquire the volumetric data, the heart and respiratory rates of mice under anesthesia are to 450 beats per minute and 55 to 65 breaths per minute,60 so the influence of the heartbeat and breathing is inevitable when acquiring volumetric data. Fig. 23D-rendered (a) PA and (b) depth-coded views from the ROI in Fig. 1(c). (c) Skin anatomy comprising the ED, PD, RD, and HD layers and vascular networks distributed differently in each skin layer. (d) PA MAP images along the direction, which corresponds to the “A” view depicted in (c). (e) Histogram representing vascular distribution depending on skin depth. The count of the histogram represents the number of pixels indicating blood vessels. (f) PA MAP images along the direction, corresponding to the “B” view in (c). (g)–(i) Depth-encoded PA MAP images corresponding to the PD, RD, and HD, which is separated with the red and blue dots in (f). ![]() Similar to the vascular characteristics in the skin anatomy, the histogram features two local maximum points, which are indicated by the red and blue points presented in Fig. 2(e). These two points respectively reflect the high vascular density in the PD and HD, which serve as the criteria for segmenting the papillary, reticular, and HD. Based on the two local maxima, the PA MAP image along the direction [e.g., “B” view shown in Fig. 2(c)] was divided into PA MAP images corresponding to the PD, RD, and HD [Figs. 2(f)–2(i)]. This volumetric segmentation yielded a volume slice at a constant distance from the skin profile using 3D PHOVIS, which is not based on the Cartesian coordinate system.46 In the PD, tiny capillaries are spread, which are depicted by the white arrows illustrated in Fig. 2(g). Moreover, a pair of relatively large arterioles and venules are present in the HD, which are represented by the white arrows presented in Fig. 2(i). The RD contains blood vessels connecting the PD and the HD, as denoted by the white arrows in Fig. 2(h). However, because of the limited-view effect in our OR-PAM system, the vertically oriented blood vessels in the RD were difficult to visualize.61,62 3.2.Time-dependent Vascular Changes in Each Skin Layer by CorticosteroidsTo investigate the vascular changes induced by corticosteroids, the blood vessels were photoacoustically monitored for 60 min at 10-min interval following the different procedures introduced in the Methods section: (1) hydrocortisone subcutaneous injection, (2) hydrocortisone topical application, (3) nonsteroidal topical application, and (4) no injection or topical application (control) (Fig. S1 in the Supplemental Material). Figure 3 shows the depth-encoded PA MAP images of the PD, RD, and HD at the time points of 0, 30, and 60 min. In the case of (1) hydrocortisone subcutaneous injection, the blood vessels in the PD and HD maintained their density without constriction; however, a decrease in the vascular density was clearly observed in the RD [Figs. 3(a) and 3(b)]. In the case of (2) hydrocortisone topical application, the vascular density decreased in all layers, and the closer they are to the skin surface, the more the vascular density decreased [Figs. 3(c) and 3(d)]. Observably, hydrocortisone-induced vasoconstriction appears in capillaries but not in large arterial and venous pairs in the middle of the ROI on the mouse thigh. This occurrence clearly reflects capillary constriction, which is the mechanism of action of corticosteroids. In contrast, for the cases of (3) nonsteroidal topical application and (4) no injection/topical application, no changes were observed in the vascular density or PA signals for all layers. Fig. 3Vascular changes based on depth-encoded PA images of the PD, RD, and HD. The first column shows the PA MAP images, the second column does the depth-encoded PA MAP images corresponding to the PD, the third column does those of the RD, and the fourth column does those of the HD. (a) and (b) PA images acquired at the time points of 0, 60 min after hydrocortisone subcutaneous injection. (c) and (d) PA images at the time points of 0, 60 min after hydrocortisone topical application. ![]() The vasoconstrictions induced by the hydrocortisone subcutaneous injection and topical application are visualized via high-resolution volumetric PA imaging. Notably, different vasoconstriction trends are observed in each skin layer, depending on the mode of dose delivery into the body. When the hydrocortisone solution is subcutaneously injected using a syringe, the blood vessels are constricted only in the RD. In contrast, in the case of hydrocortisone topical application, vasoconstriction is clearly observed in both the papillary and RD. These results imply that the hydrocortisone is absorbed into the body and acts immediately on blood vessels at the injection/application site. In contrast, vasoconstriction does not occur in the cases of nonsteroidal topical application because the anti-inflammatory mechanism of dexpanthenol differs from that of corticosteroids. Dexpanthenol acts as a precursor of coenzyme A, which serves as a cofactor in various enzyme-catalyzed reactions essential for the metabolism of steroid hormones.63–65 Consequently, the topical use of dexpanthenol induces anti-inflammatory activity in the skin by enabling the body to synthesize steroid hormones without vasoconstriction. As expected, when subcutaneous injection or topical application is not performed, no significant vascular changes are observed. 3.3.Quantitative Comparison of VasoconstrictionFurthermore, to quantify the change in vascular density, the PA MAP images are binarized using global thresholding under the assumption that the upper and lower sides in the bimodal histogram of the PA MAP image represent blood vessels and background, respectively. There are a total of 7 PA MAP images from 0 to 60 min per mouse, and because they are obtained from the same location, we apply the same threshold to each image. We used the Otsu’s method to obtain the global image threshold for each of the seven images. Subsequently, we defined the vascular density according to the number of pixels greater than the average value of the seven thresholds; this parameter was thereafter normalized by employing the first time-point data (i.e., ) to obtain the relative vascular density. Figure 4 shows the quantified vascular densities acquired from six mice in each group, which are represented as means and standard errors. The vascular densities in the PA MAP images covering all layers gradually decreases for 1 h solely upon the administration of hydrocortisone as follows: in the (1) hydrocortisone subcutaneous injection group and in the (2) hydrocortisone topical application group [Fig. 4(a)]. In contrast, no changes in vascular density occurred in the control group, which did not undergo hydrocortisone treatment: in (3) nonsteroidal topical application group and in the (4) control group. For a more detailed layer-by-layer analysis, the aforementioned method for quantifying vascular density was applied to the layered PA MAP images. In the PD closest to the skin, vasoconstriction of occurs in the (2) hydrocortisone topical application group, but the vascular density remains within 10% for the other groups [Fig. 4(b)]. In the RD, the vascular density decreases by for the (1) hydrocortisone subcutaneous injection group and by in the (2) hydrocortisone topical application group, with gradually decreasing slopes [Fig. 4(c)]. The blood vessels in the HD tended to constrict slightly () in the (2) hydrocortisone topical application group, but no significant effects on the vasculature are observed for the other groups [Fig. 4(d)]. Fig. 4Time-dependent changes in vascular densities by hydrocortisone subcutaneous injection (red line), hydrocortisone topical application (blue), nonsteroidal topical application (green), and control (black) in the (a) whole skin; (b) papillary dermis; (c) reticular dermis; and (d) hypodermis. ![]() Figure 5 shows the methodological analysis of dermatological treatment for vascular changes and the statistical analysis via a two-tailed paired -test. In the (1) hydrocortisone subcutaneous injection group, a vasoconstrictive effect is observed solely in the RD, thereby implying that the hydrocortisone subcutaneous injection exhibits the corticosteroid mechanism locally only at the injection site [Fig. 5(a)]. In contrast, in the (2) hydrocortisone topical application group, the decrease in vascular density in each layer is distinct. The closer the layer is to the skin surface, the more the vascular density decreases, which indicates that topical application of corticosteroid on the skin is gradually absorbed into the body and the direction of absorption of the topical application is inward from the skin surface [Fig. 5(b)]. No significant differences are observed in the vasculature of the (3) nonsteroidal topical application group or (4) control group, thereby quantitatively demonstrating that the anti-inflammatory mechanism of the nonsteroidal topical application is not based on vasoconstriction. The amount of vasoconstriction on each layer in the groups for 60 min and its significance through a two-tailed paired -test are plotted in Fig. 5(c). The vasoconstriction of the RD in the (1) hydrocortisone subcutaneous injection group and (4) control group are significantly different (). In addition, a vasoconstrictive effect is observed solely in the RD in the (1) hydrocortisone subcutaneous injection group, indicating a significant difference in the PD and RD (). (2) The hydrocortisone topical application group also exhibits significantly different vasoconstrictions of the papillary and RD relative to the (4) control group. Figure 5(d) shows the movement in the depth direction of the vascular network’s centroid. In the hydrocortisone topical application group, the centroid of the vascular network gradually deepens. Due to the vasoconstriction of the upper capillaries, the centroid of the vascular network moves downward. On the other hand, in the hydrocortisone subcutaneous injection group, vasoconstriction of capillaries was observed in the PA MAP image, but the centroid remains almost unchanged, implying that vasoconstriction occurred in the center of dermis near the injection site. These results conclusively imply that hydrocortisone induces vasoconstriction in the skin by the corticosteroid mechanism regardless of the administration method; however, the vasoconstriction occurs differently in each anatomical layer of the skin depending on the administration method. This in-depth methodological analysis of dermatological treatment is possible due to the volumetric PA images. Fig. 5Time-dependent changes in vascular densities by (a) hydrocortisone subcutaneous injection and (b) hydrocortisone topical application in papillary dermis (blue line), reticular dermis (green), and hypodermis (red). (c) Statistical analysis of the vasoconstriction over 60 min in the papillary dermis, reticular dermis, and hypodermis among groups. **, ; ***, ; two-tailed paired -tests. (d) Movement in the depth direction of the vascular network’s centroid by hydrocortisone subcutaneous injection (cyan) and hydrocortisone topical application (magenta). ![]() 4.Discussion and ConclusionCorticosteroids are widely used drugs for the treatment of skin diseases; however, their misuse and abuse may adversely affect patient compliance or result in side effects. Therefore, to effectively and safely treat patients with accurate prescriptions, a sufficient understanding of steroid use is crucial. Vasoconstriction, a treatment mechanism of corticosteroids, is considered to be an indicator of their effectiveness. However, indirect methods of observation, such as a change in the skin color, are currently employed in clinics and hospitals because of the absence of direct methods for evaluating vasoconstriction. In this study, we have empirically validated that OR-PAM can noninvasively visualize blood vessels without the use of contrast agents and can directly monitor vasoconstriction. However, because the maximum imaging depth of OR-PAM is limited due to optical scattering in tissues, PA monitoring with OR-PAM may be limited depending on the dermatologically treated site. In addition, to apply the proposed method to humans, the system should be further developed and spatial resolution may be sacrificed. As injected corticosteroids are typically known to be absorbed into the body and lead to systemic side effects, topical application that can be used directly on the diseased area is the preferred mode of treatment for patients with skin disease.66,67 However, the long-term use of corticosteroids through topical application may cause the capillaries to lose elasticity due to the repeated vasoconstriction mechanism. Eventually, this repeated vasoconstriction causes side effects, such as sagging of blood vessels and capillary dilatation. Therefore, injecting an appropriate amount of corticosteroid at the correct location while monitoring vasoconstriction via PA imaging may be a more beneficial strategy for mitigating these side effects. Furthermore, the side effects of long-term corticosteroid treatment can be monitored through PA imaging, which is an added advantage. Although PA imaging may be an optimal strategy for vascular monitoring, further technical advances are required to provide better clinical aid. Because PA imaging optimally represents blood vessels in volume, the skin layers can be categorized based on the characteristics of the blood vessels, but certain errors may occur. Considering that not only blood vessels but also other biological tissues are present under the skin, accurately segmenting these layers based on vascular characteristics solely obtained via PA imaging is challenging. Another imaging technique used in dermatology, optical coherence tomographic (OCT) imaging, is a specialized technique for 3D structural imaging that can better segment the skin layers.68–70 Considering their advantages, PA and OCT imaging can be utilized in tandem to yield more accurate information. Owing to the recently developed transparent US transducer, combined multi-modal PA/OCT imaging is easier to implement than ever before.71–74 Notably, such a system featuring OCT-guided PA can facilitate a higher quality of imaging and thus has great potential as an imaging tool in dermatology. In summary, we have employed OR-PAM to observe vasoconstriction, which is one of the treatment mechanisms of corticosteroids in the skin. Through high-resolution volumetric PA imaging, we have visually monitored corticosteroid-induced vasoconstriction and quantified changes in vascular density in different skin layers. Each skin layer responded differently depending on the administration method of corticosteroid: subcutaneous injection or topical application. In contrast, no vasoconstriction was observed after topical nonsteroidal application. Based on these quantitatively compared results, we suggest that high-resolution PA imaging of the skin can be a useful clinical tool for evaluating vasoconstriction induced by corticosteroids in the skin. In particular, this strategy can facilitate the prescription of personalized amounts and concentrations. DisclosuresC. K and J. Y. K have financial interests in OPTICHO, although it did not support this work. AcknowledgmentsThis research was supported by the Basic Science Research Program through National Research Foundation of Korea (NRF) funded by the Ministry of Science and ICT (Grant No. 2019R1A2C2006269) and the Ministry of Education (Grant No. 2020R1A6A1A03047902); by the National R&D Program through the National Research Foundation of Korea (NRF) funded by the Ministry of Science and ICT (Grant Nos. 2021M3C1C3097624 and 2020M3H2A1078045); by the Korea Medical Device Development Fund grant funded by the Korea Government (the Ministry of Science and ICT, the Ministry of Trade, Industry and Energy, the Ministry of Health & Welfare, the Ministry of Food and Drug Safety) (9991007019, KMDF_PR_20200901_0008); and by BK21 FOUR project. ReferencesM. B. Sulzberger, V. H. Witten and S. N. Yaffe,
“Cortisone acetate administered orally in dermatologic therapy,”
Archiv. Dermatol., 119
(10), 858
–864 https://doi.org/10.1001/archderm.1983.01650340068021
(1983).
Google Scholar
S. H. Yu et al.,
“A systematic review of the safety and efficacy of systemic corticosteroids in atopic dermatitis,”
J. Am. Acad. Dermatol., 78
(4), 733
–740.e11 https://doi.org/10.1016/j.jaad.2017.09.074 JAADDB 0190-9622
(2018).
Google Scholar
P. J. Barnes and I. M. Adcock,
“How do corticosteroids work in asthma?,”
Ann. Internal Med., 139
(5_Part_1), 359
–370 https://doi.org/10.7326/0003-4819-139-5_Part_1-200309020-00012 AIMEAS 0003-4819
(2003).
Google Scholar
M. M. Weiss,
“Corticosteroids in rheumatoid arthritis,”
Semin. Arthritis Rheumatism, 19
(1), 9
–21 https://doi.org/10.1016/0049-0172(89)90083-8
(1989).
Google Scholar
K. Kragballe,
“Topical corticosteroids: mechanisms of action,”
Acta Derm. Venereol. Suppl., 151 7
–10
(1989).
Google Scholar
B. M. Altura,
“Role of glucocorticoids in local regulation of blood flow,”
Am. J. Physiol.-Legacy Content, 211
(6), 1393
–1397 https://doi.org/10.1152/ajplegacy.1966.211.6.1393
(1966).
Google Scholar
J. D. Guin et al.,
“Quantitative vasoconstrictor assay for topical corticosteroids: the puzzling case of fluocinolone acetonide,”
J. Am. Acad. Dermatol., 29
(2, Part 1), 197
–202 https://doi.org/10.1016/0190-9622(93)70167-R JAADDB 0190-9622
(1993).
Google Scholar
P. Bjerring and P. H. Andersen,
“Skin reflectance spectrophotometry,”
Photodermatol, 4
(3), 167
–171 PPPHEW 0905-4383
(1987).
Google Scholar
L. Duteil et al.,
“Objective assessment of topical corticosteroids and non-steroidal anti-inflammatory drugs in methyl-nicotinate-induced skin inflammation,”
Clin. Exp. Dermatol., 15
(3), 195
–199 https://doi.org/10.1111/j.1365-2230.1990.tb02071.x CEDEDE 1365-2230
(1990).
Google Scholar
S. Y. Chan and A. L. W. Po,
“Quantitative skin blanching assay of corticosteroid creams using tristimulus colour analysis,”
J. Pharm. Pharmacol., 44
(5), 371
–378 https://doi.org/10.1111/j.2042-7158.1992.tb03628.x JPPMAB 0022-3573
(1992).
Google Scholar
M. Zvidzayi et al.,
“A novel approach to assess the potency of topical corticosteroids,”
Pharmaceutics, 13
(9), 1456 https://doi.org/10.3390/pharmaceutics13091456
(2021).
Google Scholar
R. B. Stoughton,
“Bioassay system for formulations of topically applied glucocorticosteroids,”
Arch. Dermatol., 106
(6), 825
–827 https://doi.org/10.1001/archderm.1972.01620150011002
(1972).
Google Scholar
R. C. Cornell,
“Clinical trials of topical corticosteroids in psoriasis: correlations with the vasoconstrictor assay,”
Int. J. Dermatol., 31 38
–40 https://doi.org/10.1111/j.1365-4362.1992.tb04012.x IJDEBB 1365-4362
(1992).
Google Scholar
L. V. Wang and J. Yao,
“A practical guide to photoacoustic tomography in the life sciences,”
Nat. Methods, 13
(8), 627
–638 https://doi.org/10.1038/nmeth.3925 1548-7091
(2016).
Google Scholar
J. Kim et al.,
“Super-resolution localization photoacoustic microscopy using intrinsic red blood cells as contrast absorbers,”
Light Sci. Appl., 8 103 https://doi.org/10.1038/s41377-019-0220-4
(2019).
Google Scholar
J. Kim et al.,
“Deep learning acceleration of multiscale superresolution localization photoacoustic imaging,”
Light Sci. Appl., 11 131 https://doi.org/10.1038/s41377-022-00820-w
(2022).
Google Scholar
S. Jeon et al.,
“Real-time delay-multiply-and-sum beamforming with coherence factor for in vivo clinical photoacoustic imaging of humans,”
Photoacoustics, 15 100136 https://doi.org/10.1016/j.pacs.2019.100136
(2019).
Google Scholar
W. Choi, D. Oh and C. Kim,
“Practical photoacoustic tomography: realistic limitations and technical solutions,”
J. Appl. Phys., 127
(23), 230903 https://doi.org/10.1063/5.0008401 JAPIAU 0021-8979
(2020).
Google Scholar
C. Lee et al.,
“Three-dimensional clinical handheld photoacoustic/ultrasound scanner,”
Photoacoustics, 18 100173 https://doi.org/10.1016/j.pacs.2020.100173
(2020).
Google Scholar
J. Kim et al.,
“Multiparametric photoacoustic analysis of human thyroid cancers in VivoPhotoacoustic analysis of human thyroid cancers,”
Cancer Res., 81
(18), 4849
–4860 https://doi.org/10.1158/0008-5472.CAN-20-3334 CNREA8 0008-5472
(2021).
Google Scholar
B. Park et al.,
“3D wide‐field multispectral photoacoustic imaging of human melanomas in vivo: a pilot study,”
J. Eur. Acad. Dermatol. Venereol., 35
(3), 669
–676 https://doi.org/10.1111/jdv.16985 JEAVEQ 0926-9959
(2021).
Google Scholar
W. Choi et al.,
“Three-dimensional multistructural quantitative photoacoustic and US imaging of human feet in vivo,”
Radiology, 303
(2), 467
–473 https://doi.org/10.1148/radiol.211029 RADLAX 0033-8419
(2022).
Google Scholar
C. Liu, Y. Liang and L. Wang,
“Single-shot photoacoustic microscopy of hemoglobin concentration, oxygen saturation, and blood flow in sub-microseconds,”
Photoacoustics, 17 100156 https://doi.org/10.1016/j.pacs.2019.100156
(2020).
Google Scholar
J. Ahn et al.,
“High-resolution functional photoacoustic monitoring of vascular dynamics in human fingers,”
Photoacoustics, 23 100282 https://doi.org/10.1016/j.pacs.2021.100282
(2021).
Google Scholar
J. Aguirre et al.,
“Assessing nailfold microvascular structure with ultra-wideband raster-scan optoacoustic mesoscopy,”
Photoacoustics, 10 31
–37 https://doi.org/10.1016/j.pacs.2018.02.002
(2018).
Google Scholar
S. Jeon et al.,
“Review on practical photoacoustic microscopy,”
Photoacoustics, 15 100141 https://doi.org/10.1016/j.pacs.2019.100141
(2019).
Google Scholar
O. Ogunlade et al.,
“Monitoring neovascularization and integration of decellularized human scaffolds using photoacoustic imaging,”
Photoacoustics, 13 76
–84 https://doi.org/10.1016/j.pacs.2019.01.001
(2019).
Google Scholar
J. W. Baik et al.,
“Super wide-field photoacoustic microscopy of animals and humans in vivo,”
IEEE Trans. Med. Imaging, 39
(4), 975
–984 https://doi.org/10.1109/TMI.2019.2938518 ITMID4 0278-0062
(2020).
Google Scholar
J. W. Baik et al.,
“Intraoperative label‐free photoacoustic histopathology of clinical specimens,”
Laser Photonics Rev., 15
(10), 2100124 https://doi.org/10.1002/lpor.202100124
(2021).
Google Scholar
J. Ahn et al.,
“Fully integrated photoacoustic microscopy and photoplethysmography of human in vivo,”
Photoacoustics, 27 100374 https://doi.org/10.1016/j.pacs.2022.100374
(2022).
Google Scholar
S.-W. Cho et al.,
“High-speed photoacoustic microscopy: a review dedicated on light sources,”
Photoacoustics, 24 100291 https://doi.org/10.1016/j.pacs.2021.100291
(2021).
Google Scholar
M. Chen et al.,
“High-speed functional photoacoustic microscopy using a water-immersible two-axis torsion-bending scanner,”
Photoacoustics, 24 100309 https://doi.org/10.1016/j.pacs.2021.100309
(2021).
Google Scholar
Q. Chen et al.,
“Progress of clinical translation of handheld and semi-handheld photoacoustic imaging,”
Photoacoustics, 22 100264 https://doi.org/10.1016/j.pacs.2021.100264
(2021).
Google Scholar
N. T. P. Truong et al.,
“Ultra-widefield photoacoustic microscopy with a dual-channel slider-crank laser-scanning apparatus for in vivo biomedical study,”
Photoacoustics, 23 100274 https://doi.org/10.1016/j.pacs.2021.100274
(2021).
Google Scholar
K. Wang et al.,
“Recent advances in high-speed photoacoustic microscopy,”
Photoacoustics, 24 100294 https://doi.org/10.1016/j.pacs.2021.100294
(2021).
Google Scholar
D. Seong et al.,
“Target ischemic stroke model creation method using photoacoustic microscopy with simultaneous vessel monitoring and dynamic photothrombosis induction,”
Photoacoustics, 27 100376 https://doi.org/10.1016/j.pacs.2022.100376
(2022).
Google Scholar
J. Yao et al.,
“High-speed label-free functional photoacoustic microscopy of mouse brain in action,”
Nat. Methods, 12
(5), 407
–410 https://doi.org/10.1038/nmeth.3336 1548-7091
(2015).
Google Scholar
J. Y. Kim et al.,
“High-speed and high-SNR photoacoustic microscopy based on a galvanometer mirror in non-conducting liquid,”
Sci. Rep., 6 34803 https://doi.org/10.1038/srep34803 SRCEC3 2045-2322
(2016).
Google Scholar
B. Lan et al.,
“High-speed widefield photoacoustic microscopy of small-animal hemodynamics,”
Biomed. Opt. Express, 9
(10), 4689
–4701 https://doi.org/10.1364/BOE.9.004689 BOEICL 2156-7085
(2018).
Google Scholar
S. Hu, K. Maslov and L. V. Wang,
“In vivo functional chronic imaging of a small animal model using optical-resolution photoacoustic microscopy,”
Med. Phys., 36
(6Part1), 2320
–2323 https://doi.org/10.1118/1.3137572 MPHYA6 0094-2405
(2009).
Google Scholar
L. Lun-De et al.,
“Investigation of the cerebral hemodynamic response function in single blood vessels by functional photoacoustic microscopy,”
J. Biomed. Opt., 17
(6), 061210 https://doi.org/10.1117/1.JBO.17.6.061210 JBOPFO 1083-3668
(2012).
Google Scholar
L. Nie et al.,
“Early-stage imaging of nanocarrier-enhanced chemotherapy response in living subjects by scalable photoacoustic microscopy,”
ACS Nano, 8
(12), 12141
–12150 https://doi.org/10.1021/nn505989e ANCAC3 1936-0851
(2014).
Google Scholar
B. Shrestha et al.,
“Photoacoustic imaging in tissue engineering and regenerative medicine,”
Tissue Eng. B Rev., 26
(1), 79
–102 https://doi.org/10.1089/ten.teb.2019.0296
(2019).
Google Scholar
B. Park et al.,
“Listening to drug delivery and responses via photoacoustic imaging,”
Adv. Drug Deliv. Rev., 184 114235 https://doi.org/10.1016/j.addr.2022.114235 ADDREP 0169-409X
(2022).
Google Scholar
C. Zhang et al.,
“Multiscale high-speed photoacoustic microscopy based on free-space light transmission and a MEMS scanning mirror,”
Opt. Lett., 45
(15), 4312
–4315 https://doi.org/10.1364/OL.397733 OPLEDP 0146-9592
(2020).
Google Scholar
S. Cho et al.,
“3D PHOVIS: 3D photoacoustic visualization studio,”
Photoacoustics, 18 100168 https://doi.org/10.1016/j.pacs.2020.100168
(2020).
Google Scholar
M. Mohesh et al.,
“High-speed simultaneous multiscale photoacoustic microscopy,”
J. Biomed. Opt., 24
(8), 086001 https://doi.org/10.1117/1.JBO.24.8.086001 JBOPFO 1083-3668
(2019).
Google Scholar
S. Hahner, S. Burger-Stritt and B. Allolio,
“Subcutaneous hydrocortisone administration for emergency use in adrenal insufficiency,”
Eur. J. Endocrinol., 169
(2), 147
–154 https://doi.org/10.1530/EJE-12-1057 EJOEEP 0804-4643
(2013).
Google Scholar
A. Rhodes et al.,
“Surviving sepsis campaign: international guidelines for management of sepsis and septic shock: 2016,”
Intensive Care Med., 43
(3), 304
–377 https://doi.org/10.1007/s00134-017-4683-6 ICMED9 0342-4642
(2017).
Google Scholar
U. R. Hengge et al.,
“Adverse effects of topical glucocorticosteroids,”
J. Am. Acad. Dermatol., 54
(1), 1
–15 https://doi.org/10.1016/j.jaad.2005.01.010 JAADDB 0190-9622
(2006).
Google Scholar
M. Kalavala et al.,
“The fingertip unit: a practical guide to topical therapy in children,”
J. Dermatol. Treat., 18
(5), 319
–320 https://doi.org/10.1080/09546630701441723
(2007).
Google Scholar
B. Zabihian et al.,
“In vivo dual-modality photoacoustic and optical coherence tomography imaging of human dermatological pathologies,”
Biomed. Opt. Express, 6
(9), 3163
–3178 https://doi.org/10.1364/BOE.6.003163 BOEICL 2156-7085
(2015).
Google Scholar
X. Li et al.,
“Multispectral raster-scanning optoacoustic mesoscopy differentiate lesional from non-lesional atopic dermatitis skin using structural and functional imaging markers,”
Photoacoustics, 28 100399 https://doi.org/10.1016/j.pacs.2022.100399
(2022).
Google Scholar
S. H. Geyer et al.,
“The dermal arteries of the human thumb pad,”
J. Anat., 223
(6), 603
–609 https://doi.org/10.1111/joa.12113 JOANAY 0021-8782
(2013).
Google Scholar
M. Schwarz et al.,
“Optoacoustic dermoscopy of the human skin: tuning excitation energy for optimal detection bandwidth with fast and deep imaging in vivo,”
IEEE Trans. Med. Imaging, 36
(6), 1287
–1296 https://doi.org/10.1109/TMI.2017.2664142 ITMID4 0278-0062
(2017).
Google Scholar
H. Yousef, M. Alhajj and S. Sharma, Anatomy, Skin (Integument), Epidermis, StatPearls Publishing, Treasure Island, Florida
(2021). Google Scholar
P. A. J. Kolarsick, M. A. Kolarsick and C. Goodwin,
“Anatomy and physiology of the skin,”
J. Dermatol. Nurses’ Assoc., 3
(4), 203
–213 https://doi.org/10.1097/JDN.0b013e3182274a98
(2011).
Google Scholar
O. Arda, N. Göksügür and Y. Tüzün,
“Basic histological structure and functions of facial skin,”
Clin. Dermatol., 32
(1), 3
–13 https://doi.org/10.1016/j.clindermatol.2013.05.021
(2014).
Google Scholar
T. M. Brown, K. Krishnamurthy,
“Histology, dermis,”
StatPearls [Internet], Princeton University, Princeton, New Jersey
(2021). Google Scholar
A. J. Ewald, Z. Werb and M. Egeblad,
“Monitoring of vital signs for long-term survival of mice under anesthesia,”
Cold Spring Harbor Protoc., 2011
(2), pdb.prot5563 https://doi.org/10.1101/pdb.prot5563
(2011).
Google Scholar
H.-C. Hsu et al.,
“Dual-axis illumination for virtually augmenting the detection view of optical-resolution photoacoustic microscopy,”
J. Biomed. Opt., 23
(7), 076001 https://doi.org/10.1117/1.JBO.23.7.076001 JBOPFO 1083-3668
(2018).
Google Scholar
J. Yao et al.,
“Clinically-translatable high-fidelity photoacoustic tomography enhanced by virtual point sources,”
(2021). Google Scholar
F. Ebner et al.,
“Topical use of dexpanthenol in skin disorders,”
Am. J. Clin. Dermatol., 3
(6), 427
–433 https://doi.org/10.2165/00128071-200203060-00005
(2002).
Google Scholar
R. J. Williams and D. H. Saunders,
“The effects of inositol, crystalline vitamin B(1) and “pantothenic acid” on the growth of different strains of yeast,”
Biochem. J., 28
(5), 1887
–1893 https://doi.org/10.1042/bj0281887
(1934).
Google Scholar
E. Proksch et al.,
“Topical use of dexpanthenol: a 70th anniversary article,”
J. Dermatol. Treat., 28
(8), 766
–773 https://doi.org/10.1080/09546634.2017.1325310
(2017).
Google Scholar
C. N. McGhee, S. Dean and H. Danesh-Meyer,
“Locally administered ocular corticosteroids,”
Drug Saf., 25
(1), 33
–55 https://doi.org/10.2165/00002018-200225010-00004 DRSAEA 0114-5916
(2002).
Google Scholar
O. Toktas et al.,
“A novel first-line treatment alternative for noncomplicated idiopathic granulomatous mastitis: combined intralesional steroid injection with topical steroid administration,”
Breast Care, 16
(2), 181
–187 https://doi.org/10.1159/000507951
(2021).
Google Scholar
J. Welzel,
“Optical coherence tomography in dermatology: a review,”
Skin Res. Technol., 7
(1), 1
–9 https://doi.org/10.1034/j.1600-0846.2001.007001001.x
(2001).
Google Scholar
T. Gambichler et al.,
“Applications of optical coherence tomography in dermatology,”
J. Dermatol. Sci., 40
(2), 85
–94 https://doi.org/10.1016/j.jdermsci.2005.07.006 JDSCEI 0923-1811
(2005).
Google Scholar
M. Ulrich et al.,
“Dynamic optical coherence tomography in dermatology,”
Dermatology, 232
(3), 298
–311 https://doi.org/10.1159/000444706 DERMEI 0742-3217
(2016).
Google Scholar
A. Dangi, S. Agrawal and S.-R. Kothapalli,
“Lithium niobate-based transparent ultrasound transducers for photoacoustic imaging,”
Opt. Lett., 44
(21), 5326
–5329 https://doi.org/10.1364/OL.44.005326 OPLEDP 0146-9592
(2019).
Google Scholar
H. Chen et al.,
“Optical-resolution photoacoustic microscopy using transparent ultrasound transducer,”
Sensors, 19
(24), 5470 https://doi.org/10.3390/s19245470 SNSRES 0746-9462
(2019).
Google Scholar
J. Park et al.,
“Quadruple ultrasound, photoacoustic, optical coherence, and fluorescence fusion imaging with a transparent ultrasound transducer,”
Proc. Natl. Acad. Sci., 118
(11), e1920879118 https://doi.org/10.1073/pnas.1920879118
(2021).
Google Scholar
B. Park et al.,
“A photoacoustic finder fully integrated with a solid-state dye laser and transparent ultrasound transducer,”
Photoacoustics, 23 100290 https://doi.org/10.1016/j.pacs.2021.100290
(2021).
Google Scholar
BiographyDonggyu Kim received his BS degree in Mechanical Engineering from the Pohang University of Science and Technology (POSTECH), Republic of Korea, in 2021. He is currently pursuing a PhD (MS-PhD joint program) in Convergence IT Engineering at POSTECH. His research interests include the development of transparent ultrasound transducer and novel biomedical imaging techniques including photoacoustic microscopy and ultrasound imaging. Joongho Ahn received his PhD in Convergence IT Engineering at Pohang University of Science and Technology (POSTECH), Republic of Korea. He was a postdoctoral research associate in the Department of Convergence IT Engineering at POSTECH and he is currently a research assistant professor at the Department of Convergence IT Engineering at POSTECH. His research interests include the development of ultrasound and photoacoustic imaging systems, and their translational research and commercialization. Eunwoo Park received his BS and MS degrees in Mechanical Engineering from Dankook University, Republic of Korea, in 2016 and 2017, respectively. He was a research associate at Advanced Photonics Research Institute, GIST (2018–2021). He is currently pursuing a PhD in convergence IT engineering at POSTECH, Republic of Korea. His research interests focus on developing novel biomedical imaging systems. Jin Young Kim received his PhD and postdoctoral training from the Departments of Mechanical Engineering and Convergence IT Engineering at Pohang University of Science and Technology (POSTECH), Republic of Korea. He is currently a research assistant professor in the Department of Convergence IT Engineering at POSTECH, working on novel photoacoustic imaging, and multimodal optical imaging systems, and their clinical applications and translations. Chulhong Kim earned his PhD and did postdoctoral training at Washington University in St. Louis, Missouri. He currently holds the Young Distinguished Professorship and Mueunjae Chair Professorship as Professor of Electrical Engineering, Convergence IT Engineering, Mechanical Engineering, and Medical Science and Engineering. He directs the Medical Device Innovation Center at Pohang University of Science and Technology in the Republic of Korea. |
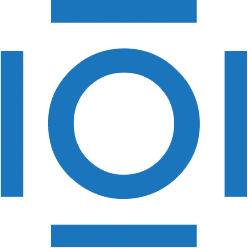
CITATIONS
Cited by 2 scholarly publications.
Skin
Blood vessels
Biological imaging
Imaging systems
Photoacoustic spectroscopy
In vivo imaging
Capillaries